Welcome to the
Nanotechnology in Sustainable Energy Laboratory at SUNY OSWEGO
Introduction
The advancement in nanoscience research over the last three decades is comparable to the industrial revolution of the eighteenth to nineteenth century. Through control of material systems in the nanometer length scale far-reaching outcomes for the 21st century are envisioned in both scientific knowledge and a wide range of technologies, including, healthcare, conservation of materials and energy, biology, environment, and education.
Incorporation of nanoscience research in the undergraduate curriculum in Universities to prepare workforce to meet the 21st century nanoscience challenge is, therefore, essential.
At the Nanotechnology in Sustainable Energy Laboratory at SUNY Oswego we specialize in the growth of compound nanostructures, optical and structural characterization of the nanostructures, Electrophoretic Assembly of nanoparticle heterostructures, Lithium Ion Battery and Sodium Ion Battery fabrication with environmentally benign and low carbon footprint Iron Oxide nanoparticle anodes.
Synthesis of Nanoparticles: When the physical dimensions of materials are in the nanometer size they exhibit tunable optical, mechanical, electronic and magnetic properties that are of interest from the point of view of both fundamental physics and technological applications like sustainable energy, biotechnology and computing. We grow nanoparticles (nps) of well controlled sizes and shapes in our Nanolab at SUNY Oswego through the colloidal synthesis method by injecting molecular precursors into hot coordinating solvents. Through precise control of injection temperature, precursor/coordinating solvent ratio, and post injection annealing we grow nanoparticles of precise size, shape and stoichiometry.
The Nanolab at SUNY Oswego is equipped with the complete range of equipment for such chemical synthesis of nanomaterials. The equipment list include, Glovebox with continuous flow of Argon gas, Schlenk lines for inert synthesis, optical diagnostic equipment and X-Ray Diffractometer for crystallinity determination.
Specific examples of our nanomaterial synthesis are:
Semiconductor Nanoparticles: We are specialized in the synthesis of nanoparticles of wide band gap semiconductors, such as Cadmium Selenide (CdSe), Cadmium Telluride (CdTe), Cadmium Sulfide (CdS), and Lead Sulfide (PbSe). These wide bandgap semiconductor materials are applicable in a wide range of technologies including solar panel, fluorescence, light emitting diodes and biotechnological imaging. We are in the process of adding nanoparticles of Silver Sulfide (Ag2S) and Copper Sulfide (Cu2S) – nanoparticles that are environmentally friendly – to our repertoire.
Metal Nanoparticles: We have successfully synthesized Cobalt (Co) nanoparticles. These magnetic metals have applications in Lithium Ion Batteries through conversion into Cobalt Oxide (Co3O4) nanoparticles.
Oxide Nanoparticles: We have successfully synthesized Aluminum Oxide (Al2O3) and Iron Oxide (Fe3O4) nanoparticles using surface stabilizing agents such as Na(AOT) molecules as the inverse micelle. Aluminum Oxide (Al2O3) is expected to find applications in electronics, optoelectronics, catalysis and thin film coatings. Superparamagnetic Iron Oxide Nanoparticles (SPION) are being used in numerous in vivo biotechnological applications such as magnetic resonance imaging (MRI) contrast enhancement, tissue repair, immunoassay, detoxification of biological fluids, hyperthermia, drug delivery and in cell separation. These applications, however, require precise control of the surface chemistry, and nanoparticle size and shapes. Using a combination of our synthesis techniques and, optical and crystallinity diagnostic tools we have achieved such precision in our oxide nanoparticle synthesis.
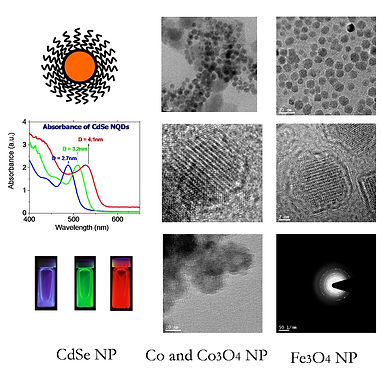
Fabrication of Nanoparticle Films Using Electrophoretic Deposition:
While the properties of individual nanoparticles are indeed fascinating to harness these properties for technological applications requires large scale, durable and patternable assembly of these nanometer size species. Conventional assembly methods - spin cast, dry cast and dip coat, with and without ligand exchange - result, in most cases, in mechanically fragile films with weak interparticle interactions. When used in fabricating devices like solar cells, these films subject charge carriers to tortuous paths and several interfaces from their generation to the electrode, leading to low efficiency.
A strong alternative to the current methods of np film deposition in the form of Electrophoretic Deposition (EPD) has emerged over the last few years and has already progressed significantly in addressing the problems associated with large-scale and well-controlled assembly of nps. Several research groups, including ours, have already demonstrated the tremendous versatility of EPD in fabricating np assemblies on a wide range of substrates including, but not limited to, Au, stainless steel, ITO and FTO. We have electrophoretically deposited nps on both flat conducting surfaces and patterned electrodes with feature sizes laterally from centimeters to as low as one micron, and thicknesses from few microns to as low as few tens of nanometers.
A typical configuration of EPD is shown in the Figure to the right where the application of DC voltage between two electrodes submerged in a np solution is used to attract and to eventually deposit them onto the electrodes.
While the potential of EPD for fabricating durable assembly of nanomaterials that are scalable and repeatable has been demonstrated by several research groups, and that the method has proven to be efficient and sustainable in the use of energy and materials, a systematic study of EPD is notably lacking. Our literature survey reveals that nearly all research groups are practicing EPD in an ad hoc manner, focusing almost exclusively on the end product, namely the films fabricated by EPD. For generalizing EPD for fabricating nanomaterial assemblies in general for industrial applications, a systematic study of the method itself is warranted. At SUNY Oswego we are engaged in a systematically and rigorously study the EPD method, the electrical nature of the interfaces produced in the electrophoretically deposited np films, and the eventual application of the films in np-based photovoltaic devices.
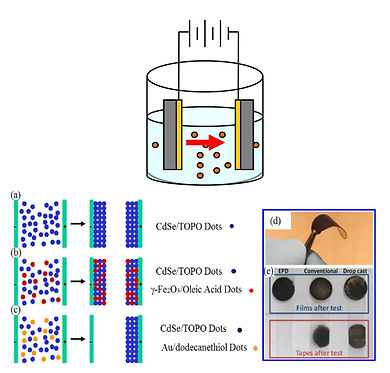
Lithium Ion Batteries: Rechargeable Lithium Ion Batteries (LIBs) are poised to be the main source of energy for the mobile lifestyle of the 21st century owing to these devices’ high energy density, portability, and simplicity. However, to be truly useful in mobile technology LIBs should possess additional characteristics of low weight, fast and efficient charge/discharge processes, and long cycle lives. Current LIB technology based on electrodes composed of bulk materials lack many of these essential characteristics. Efforts are underway for the application of nanomaterials in LIBs.
At the Nanolab in SUNY Oswego we have a comprehensive program of LIB fabrication, testing and optimization. We describe below the specifics of our LIB program.
In our nanomaterial growth facility, we chemically synthesize Cobalt (Co) nanoparticles using cobalt salts and organics surfactants. We fabricate high quality films of these nanoparticles using Electrophoretic Deposition (EPD). The Co nanoparticle films are converted into hollow Cobalt Oxide (Co3O4) nanoparticles by annealing at high temperature in the ambient. :
The Cobalt oxide nanoparticle films are used as the Anode of LIBs and Lithium foils are used as the counter electrode in a coin cell configuration. We use metal disk cutters and coin cell battery crimper from MTI company for precise sizing, electrode cutting and battery sealing.
We use Arbin Instruments Battery Tester Model 2000 to test the electrochemical properties of our Lithium Ion batteries. We discharge and charge the batteries at different current rates – from tens of microamps to ½ of an amp - and determine the capacities, specific capacities, and capacity retention of the batteries.
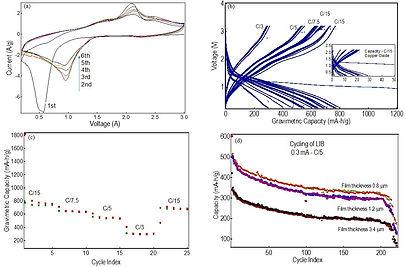
Raman Spectroscopic Analysis of Co3O4 Nanoparticle Anodes in H-LIBs: Raman spectroscopy has been found to be advantageous for materials characterization over other diagnostic tools like TEM, XRD, XPS and photoluminescence due to the facts that the former method is noninvasive and nondestructive, requires little or no sample preparation steps, can be performed either in the ambient or at the same conditions at which a material is to be used in a typical application, and finally, it does not require the material to possess a specific bandgap. Accordingly, Raman scattering has been widely used in identifying structural and electronic phases in solids, thin films, and nanostructured semiconductor and oxide materials, since scattering by phonons and electronic degrees of freedom together provide unique signatures of atomic, polar and electronic ordering.
Despite its wide range of potentials reports on Raman spectroscopic studies in battery technology is few and far between. Nanoscale unique properties, e.g., quantum confinement, surface strain, impurities, etc., are extremely important for battery performance and these are the same properties that can be studied extensively with Raman spectroscopy. We performed Raman studies on the Co3O4 nanoparticle anodes at the following stages of the battery cycling: the pristine Co3O4 nanoparticle anodes, after the first discharge of the battery, after first discharge/first charge cycle, after 25 cycles of discharge/charge at various rates, and after 100 cycles of discharge/charge. In summary, our studies show clearly that Co3O4 nanoparticles convert to elemental Co and LiO2 when the battery discharges. However, our results also indicate that the nanoparticles may not completely reconstitute back to their initial spherical shapes upon re-charging, a result that is of significance but has so far received scant attention.

Density Functional Design of Ferrite Nanoparticles for Rechargeable Battery Application: First-principle calculations based on Density Functional Theory have shifted the paradigm in experimental methodology tremendously over the last couple of decades. Instead of following the ad hoc methods of testing out different potential material systems directly in experiments, smart material predictive simulations based on DFT calculations have proven to cut down the number of experiments to be performed resulting in rapid realization of technology and devices.
We carry out predictive simulations to zero in on a subset of MnFe3-nO4 (M = Al, Fe, Co, Ni, and Cu) material systems with the correct oxidation reduction voltage, gravimetric and volumetric capacities and volume expansion/contraction due to conversion reactions for NIB application.
DFT calculations are performed under periodic boundary conditions as implemented in the Quantum Espresso code. The first calculations are performed on the prototype Fe3O4 with the initial input – the lattice constant, atomic positions and types - from the Materials Genome project. The system is allowed to relax until the force between neighboring atoms is less than a threshold value (to be determined). This results in equilibrium volume of the system. Following this, a set of battery reactions are built as the end product. Voltage plateaus and the equilibrium volumes of the products for each reaction are determined through DFT. The calculated voltage plateaus are compared with experimentally determined values from CV measurements to determine the feasibilities of the conversion reactions. Comparison of the equilibrium volumes between the reactants and the products will yield the rate of volume expansion.
For the general MnFe3-nO4 material system the distribution of the atoms over the
octahedral/tetrahedral sites are performed within the special quasirandom structure (SQR) model which ensures that the most physically relevant atomic coordinate tend toward random occupation.
All calculations are performed spin polarized within the scalar relativistic approximation. To account for the highly correlated 3d orbitals of Fe, Co and Mn the calculations will be performed at the DFT + U level using the PBE gradient corrected exchange correlation functional.
Phonon structures of the MnFe3-nO4 systems are further calculated through DFT. For each system DFT calculations will be carried out on the relaxed unit cell and on the unit cell with expanded lattice parameter to simulate the lattice expansion in nanoparticles as seen in previously on CeO2 and BaTiO3.
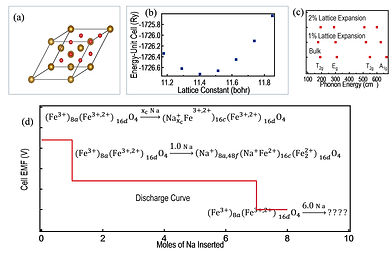